When we look around, we are met with an astonishing array of living organisms. From the plants in our gardens to the insects flitting about, from the birds chirping in the trees to our beloved pets, life manifests itself in myriad forms. Beyond what we can observe with the naked eye, there exists a world teeming with microscopic organisms, each playing a vital role in the intricate web of life. The diversity of living organisms becomes even more pronounced when we expand our observations beyond our immediate surroundings.
For instance, a journey through a dense forest reveals a staggering variety of species, each uniquely adapted to its environment. This rich diversity of life is encapsulated in the concept of biodiversity, which refers to the vast number and variety of species that inhabit our planet. Scientists estimate that between 1.7 and 1.8 million species have been identified and described, though the actual number is likely much higher as new species are continuously being discovered, even in regions thought to be well-explored.
Table of Contents
The Importance of Nomenclature: A Universal Language for Biology
Given the immense variety of life, it is crucial to have a standardized system for naming organisms. Imagine the confusion that would arise if every region or community used its local names for the same species. Communication about organisms would be nearly impossible across different areas, leading to misunderstandings and inaccuracies. This is where the concept of nomenclature comes into play.
Nomenclature is the process of assigning names to organisms in a standardized manner. This system ensures that each organism has a unique name that is recognized and used by scientists around the world. However, nomenclature is not merely about naming; it is deeply intertwined with the accurate identification and description of organisms. Only when an organism is correctly identified and described can it be assigned a scientific name. This scientific naming system follows strict rules and conventions that have been established by the scientific community.
For plants, the rules for naming are provided by the International Code for Botanical Nomenclature (ICBN). For animals, the corresponding guidelines are set by the International Code of Zoological Nomenclature (ICZN). These codes ensure that each species has a unique scientific name and that no two species share the same name. The adoption of these universal codes allows biologists around the world to communicate clearly and accurately about the species they study.
Binomial Nomenclature: The System of Two Names
One of the most significant contributions to the field of taxonomy was made by the Swedish botanist Carl Linnaeus. He introduced the system of Binomial Nomenclature, a method of naming organisms using two components: the Generic name and the Specific epithet. This system has become the foundation of modern taxonomy and is used by biologists worldwide.
To understand binomial nomenclature, consider the example of the mango. The scientific name for the mango is Mangifera indica. In this name, Mangifera represents the genus, and Indica is the specific epithet, which refers to the particular species within the genus. The name is written in italics to signify its Latin origin, and the first letter of the genus is always capitalized, while the specific epithet is written in lowercase.
Some other examples of binomial nomenclature include:
- Homo sapiens: The scientific name for humans, where Homo is the genus, and sapiens is the species.
- Panthera Leo: The scientific name for the lion, with Panthera as the genus and Leo as the species.
- Rosa indica: The scientific name for a type of rose, with Rosa as the genus and indica as the species.
It is also customary to include the name of the scientist who first described the species in the scientific name. For instance, in the name Mangifera indica Linn., “Linn.” stands for Linnaeus, indicating that he was the first to describe the mango species.
The Need for Classification: Organizing the Diversity of Life
Given the vast diversity of life, it is nearly impossible to study all living organisms without a system for organizing them into groups. This process is known as classification. Classification involves grouping organisms into categories based on shared characteristics, making it easier to study and understand them.
Classification begins with broad categories and moves towards more specific ones. For example, at the highest level, all living organisms can be classified into one of the five kingdoms: Monera, Protista, Fungi, Plantae, and Animalia. Within each kingdom, organisms are further classified into phyla, classes, orders, families, genera, and species. This hierarchical system allows scientists to systematically categorize and study the diversity of life.
To illustrate, consider the classification of the domestic dog:
- Kingdom: Animalia
- Phylum: Chordata
- Class: Mammalia
- Order: Carnivora
- Family: Canidae
- Genus: Canis
- Species: Canis lupus
- Subspecies: Canis lupus familiaris
This classification not only helps in identifying the dog but also provides insight into its evolutionary relationships with other animals, such as wolves (Canis lupus), which belong to the same species but different subspecies.
Similarly, in the plant kingdom, wheat can be classified as follows:
- Kingdom: Plantae
- Phylum: Angiosperms
- Class: Monocotyledons
- Order: Poales
- Family: Poaceae
- Genus: Triticum
- Species: Triticum aestivum
This classification highlights the shared characteristics of wheat with other cereals like rice and maize, all of which belong to the Poaceae family.
Taxonomy: The Science of Classification
Taxonomy is the branch of biology that deals with the identification, nomenclature, and classification of organisms. It is a systematic approach to understanding the relationships between different species and organizing them into groups. The process of taxonomy involves several steps, including characterization, identification, classification, and nomenclature.
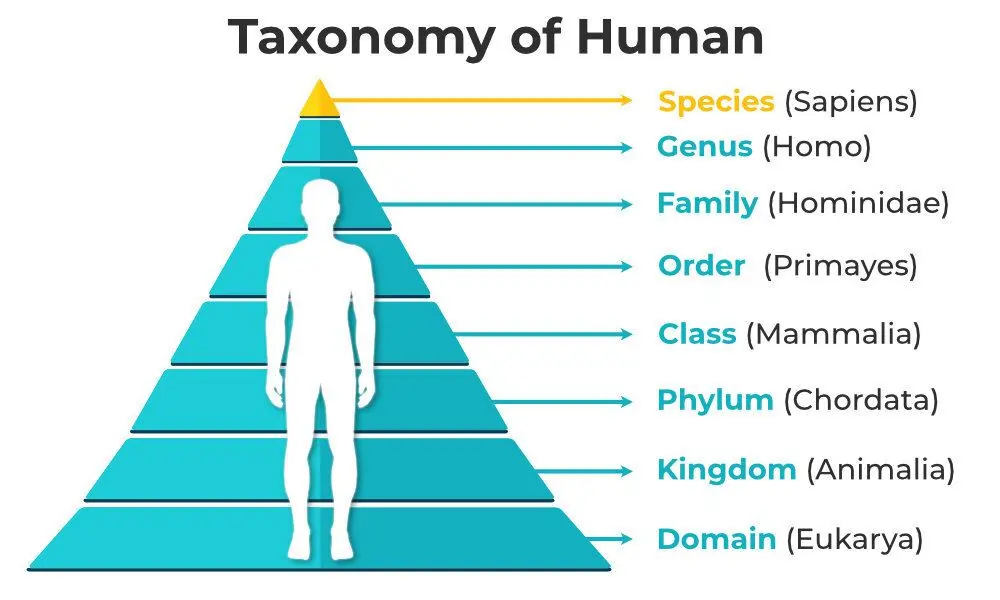
- Characterization: This involves studying the observable features of an organism, both external and internal, to understand its unique traits.
- Identification: Once the organism’s characteristics are known, it is identified by comparing its traits with known species.
- Classification: After identification, the organism is classified into the appropriate taxonomic categories based on its characteristics and evolutionary relationships.
- Nomenclature: Finally, the organism is given a scientific name according to the rules of binomial nomenclature.
Modern taxonomy also incorporates molecular data, such as DNA sequencing, to provide more accurate classifications based on evolutionary relationships. For instance, DNA barcoding is a technique used to identify species based on a short, standardized region of genetic material. This has become an essential tool in taxonomy, especially for identifying species that are difficult to distinguish based on physical characteristics alone.
Systematics: Understanding the Relationships Among Organisms
Systematics is a broader field that encompasses taxonomy but also focuses on understanding the evolutionary relationships among organisms. The term “systematics” is derived from the Latin word “systema,” meaning a systematic arrangement of organisms. Systematics not only involves the identification, classification, and nomenclature of organisms but also examines their evolutionary history and the connections between different species.
The study of systematics allows scientists to construct phylogenetic trees, which are diagrams that represent the evolutionary relationships among various species. These trees help biologists trace the lineage of species and understand how they have evolved. For example, phylogenetic analysis has shown that birds are more closely related to certain groups of dinosaurs than to reptiles, a finding that has revolutionized our understanding of avian evolution.
Systematics also plays a crucial role in conservation biology. By understanding the evolutionary relationships among species, conservationists can prioritize efforts to protect biodiversity. For instance, preserving a species that represents a unique branch on the tree of life is often considered more critical than conserving a species with many close relatives.
Conclusion
The diversity of life on Earth is truly remarkable, and understanding this diversity is essential for both scientific research and the preservation of our natural world. Through the processes of nomenclature, classification, taxonomy, and systematics, biologists have developed a comprehensive framework for studying and understanding the vast array of living organisms. These systems not only help scientists communicate effectively but also provide valuable insights into the evolutionary relationships among species. As new species continue to be discovered, and as our understanding of life deepens, the study of biodiversity will remain a central and dynamic field in biology.
Frequently Asked Questions (FAQs)
What is biodiversity, and why is it important?
Biodiversity refers to the variety of life forms on Earth, including the different species of plants, animals, fungi, and microorganisms, as well as the genetic diversity within these species and the ecosystems they form. It encompasses the full range of species from the tiniest bacteria to the largest mammals and everything in between.
Biodiversity is crucial for several reasons:
- Ecological Balance: Biodiversity helps maintain the balance of ecosystems, ensuring that they function effectively. Each species plays a unique role, such as pollination, decomposition, water purification, and soil fertility, which are all vital processes for the survival of ecosystems.
- Resilience: Diverse ecosystems are more resilient to environmental changes and disturbances. For example, if a particular species is affected by disease, a diverse ecosystem can adapt and continue to thrive because other species can fulfill similar roles.
- Human Well-being: Biodiversity is directly linked to human health and survival. It provides us with food, medicine, clean water, and raw materials for industry. Many of the medicines we use today are derived from plant and animal products.
- Cultural Significance: Biodiversity also has cultural, spiritual, and aesthetic value. Many cultures around the world are deeply connected to nature and biodiversity, which is reflected in their traditions, rituals, and lifestyles.
Unfortunately, human activities such as deforestation, pollution, and climate change are leading to the loss of biodiversity at an alarming rate. Protecting biodiversity is essential for sustaining life on Earth and ensuring the well-being of future generations.
What is taxonomy, and how does it help in the study of living organisms?
Taxonomy is the branch of biology that deals with the classification, naming, and identification of living organisms. It involves organizing organisms into hierarchical categories based on shared characteristics and evolutionary relationships, making it easier to study and understand the immense diversity of life.
Taxonomy helps in several ways:
- Organization: Taxonomy provides a structured way to categorize organisms, making it easier to study and reference them. Without taxonomy, the vast number of species would be overwhelming to manage.
- Communication: By assigning standardized names to organisms, taxonomy allows scientists around the world to communicate effectively. For instance, the scientific name Homo sapiens is universally recognized as referring to humans, regardless of language or region.
- Identification: Taxonomy provides tools and methods for identifying organisms based on their characteristics. This is crucial for many fields, such as ecology, agriculture, and medicine, where identifying species accurately can have significant implications.
- Understanding Evolution: Taxonomy is closely linked to evolutionary biology. By studying the relationships between different groups of organisms, taxonomists can trace the evolutionary history of life on Earth. This helps us understand how species have evolved and how they are related to one another.
- Conservation: Taxonomy plays a key role in conservation biology by helping to identify species that are at risk of extinction. It also helps prioritize conservation efforts by identifying species that are evolutionarily unique and therefore of high conservation value.
In summary, taxonomy is a foundational science that underpins our understanding of biodiversity and the relationships among living organisms.
What is binomial nomenclature, and why is it used?
Binomial nomenclature is a formal system of naming species using two Latin or Latinized words. This system was developed by the Swedish botanist Carl Linnaeus in the 18th century and is still used by scientists today. The two components of a binomial name are:
- Generic name (Genus): The first part of the name represents the genus to which the species belongs. It is always capitalized.
- Specific epithet (Species): The second part of the name represents the specific species within the genus. It is not capitalized.
For example, in the scientific name Homo sapiens:
- Homo is the genus name.
- sapiens is the specific epithet, identifying the particular species within the genus Homo.
Binomial nomenclature is used because:
- Uniqueness: Each species has a unique binomial name, which helps prevent confusion. For instance, the common name “pine” can refer to several different species of trees, but the scientific name Pinus sylvestris refers to a specific species of pine.
- Universality: Scientific names are recognized globally, regardless of local languages. This universality allows scientists from different parts of the world to communicate clearly about specific species.
- Precision: Binomial names provide more precision than common names, which can vary widely and may be used for multiple species. For example, “panther” can refer to both a leopard and a cougar, but the scientific names Panthera pardus and Puma concolor distinguish between these two distinct species.
- Reflects Relationships: The binomial system also reflects the evolutionary relationships between species. The genus name groups species that are closely related, indicating that they share a common ancestor.
Overall, binomial nomenclature is a standardized, precise, and universally accepted method of naming species, making it an essential tool in biology.
How are scientific names assigned to organisms?
Scientific names are assigned to organisms based on a set of standardized rules and conventions established by international codes of nomenclature. These rules ensure that each species has a unique and universally recognized name. The process of assigning scientific names involves several steps:
- Discovery and Identification: When a new species is discovered, scientists first identify its unique characteristics and compare them with known species to confirm that it is indeed a new species.
- Description: The species is then formally described in a scientific publication. This description includes detailed information about the organism’s physical characteristics, habitat, behavior, and any other relevant details. The description must be thorough enough to distinguish the species from all other known species.
- Naming: The scientist who describes the species assigns it a binomial name following the rules of binomial nomenclature. The first part of the name (the genus) is capitalized, and the second part (the specific epithet) is in lowercase. Both parts are italicized or underlined. The name may be derived from Latin, or it can be Latinized from other languages.
- Publication and Validation: The new name and description are published in a scientific journal or a recognized publication. The name becomes official only after it has been published according to the rules set by the relevant international code (e.g., the International Code of Botanical Nomenclature for Plants or the International Code of Zoological Nomenclature for Animals).
- Author Citation: The name of the person who first described the species is often included after the binomial name in an abbreviated form. For example, in Homo sapiens Linnaeus, “Linnaeus” refers to Carl Linnaeus, who first described the species.
- Priority: If two names are accidentally assigned to the same species, the name that was published first (according to the rules) takes priority, and the later name is considered a synonym.
- Type Specimen: A “type specimen” is designated as the reference example of the species. This specimen is usually kept in a museum or herbarium and serves as the standard for future comparisons.
The process of assigning scientific names is rigorous and follows international guidelines to ensure consistency, accuracy, and stability in the naming of organisms.
What is classification, and how is it different from taxonomy?
Classification is the process of organizing living organisms into groups or categories based on shared characteristics. It involves sorting species into a hierarchical structure that includes broad groups like kingdoms and phyla, down to more specific groups like genera and species. Classification is a fundamental aspect of taxonomy, but it focuses specifically on the grouping and categorization of organisms.
Taxonomy, on the other hand, is a broader discipline that encompasses classification, identification, nomenclature (naming), and the study of evolutionary relationships. While classification deals with how organisms are grouped, taxonomy also involves the rules and procedures for naming these groups and understanding the relationships between them.
Key Differences:
- Scope: Classification is a subset of taxonomy. Taxonomy includes classification as well as the principles and processes of naming organisms (nomenclature) and identifying them.
- Function: Classification primarily deals with organizing organisms into hierarchical categories. Taxonomy is concerned with the entire process of categorizing, naming, and understanding the evolutionary connections among organisms.
- Process: Classification involves placing organisms into groups based on their similarities and differences. Taxonomy includes the classification process but also involves naming (using binomial nomenclature) and determining the relationships among different groups.
Example:
- In classification, humans are grouped into the kingdom Animalia, phylum Chordata, class Mammalia, order Primates, family Hominidae, genus Homo, and species Homo sapiens.
- Taxonomy not only includes this classification but also provides the rules for naming humans as Homo sapiens and studying their relationship with other primates.
In summary, while classification is about grouping organisms, taxonomy is a comprehensive field that includes classification along with naming and studying the evolutionary history of these groups.
What are the major levels of classification in biology?
The major levels of classification in biology, also known as taxonomic ranks, form a hierarchical structure that organizes living organisms into increasingly specific categories. These ranks are:
- Domain: The highest and most inclusive rank. All life is divided into three domains:
- Archaea: Consists of prokaryotic organisms that often live in extreme environments.
- Bacteria: Includes the majority of prokaryotic organisms, which are found in a wide range of habitats.
- Eukarya: Encompasses all eukaryotic organisms, including plants, animals, fungi, and protists.
- Kingdom: The next level, which groups organisms based on fundamental similarities in their cell structure and mode of nutrition. The five widely recognized kingdoms are:
- Monera: Consists of prokaryotic organisms (bacteria and archaea).
- Protista: A diverse group of eukaryotic organisms, mostly unicellular.
- Fungi: Eukaryotic organisms that absorb nutrients from organic materials.
- Plantae: Multicellular, eukaryotic organisms that produce their own food through photosynthesis.
- Animalia: Multicellular, eukaryotic organisms that obtain food by ingesting other organisms.
- Phylum: Groups organisms within a kingdom based on major body plans and structural features. For example, the phylum Chordata includes animals with a notochord, such as vertebrates.
- Class: Further divides organisms within a phylum. For example, the class Mammalia within the phylum Chordata includes all mammals.
- Order: Groups organisms within a class based on more specific characteristics. For instance, the order Carnivora includes mammals that are primarily meat-eaters.
- Family: Groups organisms within an order that share even more specific characteristics. For example, the family Felidae includes cats.
- Genus: A more specific grouping within a family. Organisms within a genus share very closely related characteristics. For instance, Panthera is a genus that includes big cats like lions and tigers.
- Species: The most specific level of classification. A species is a group of organisms that can interbreed and produce fertile offspring. For example, Homo sapiens is the species name for humans.
- Subspecies: An additional rank below species, used to identify populations within a species that have distinct characteristics but can still interbreed. For example, Canis lupus familiaris refers to domestic dogs, a subspecies of the gray wolf (Canis lupus).
These levels of classification provide a framework for organizing and understanding the vast diversity of life on Earth, from the broadest similarities to the most specific differences.
What is a phylogenetic tree, and how is it used in systematics?
A phylogenetic tree, also known as an evolutionary tree, is a diagram that represents the evolutionary relationships among different species or other taxonomic groups. The tree is constructed based on the principle that all life shares a common ancestor, and it visually depicts how species have diverged from this common ancestor over time.
Components of a Phylogenetic Tree:
- Root: The base of the tree, representing the common ancestor of all the species included in the tree.
- Branches: Lines that extend from the root, representing evolutionary lineages. Each branch point, or node, represents a divergence event where a single lineage split into two or more distinct lineages.
- Leaves or Tips: The ends of the branches, representing the current species or groups.
- Clade: A group of organisms that includes an ancestor and all its descendants. A clade is also known as a monophyletic group.
Uses in Systematics:
- Understanding Evolutionary Relationships: Phylogenetic trees help scientists visualize the evolutionary relationships among species. By comparing the structure of the tree, scientists can determine which species are more closely related to each other and infer the sequence of evolutionary events.
- Tracing Lineages: The tree shows the path of evolution from a common ancestor to the various descendant species. This helps in understanding how certain traits evolved and how species have adapted to different environments.
- Classification: Phylogenetic trees are used to inform taxonomic classification. By understanding the evolutionary relationships, scientists can classify organisms in a way that reflects their shared ancestry.
- Predicting Traits: The tree can be used to predict traits of ancestral species or unstudied species by examining the traits of closely related species on the tree.
- Conservation Prioritization: In conservation biology, phylogenetic trees help prioritize species for conservation efforts. Species that represent unique branches on the tree (i.e., those with few close relatives) may be prioritized because their extinction would result in a disproportionate loss of evolutionary history.
Phylogenetic trees are constructed using various types of data, including morphological traits, genetic sequences, and molecular markers. Advances in DNA sequencing technologies have greatly enhanced the accuracy and resolution of phylogenetic trees, making them an essential tool in modern systematics.
What is the difference between homologous and analogous structures?
Homologous structures and analogous structures are terms used to describe similarities in the anatomy of different species, but they arise from different evolutionary processes and have different implications for understanding evolutionary relationships.
Homologous Structures:
- Definition: Homologous structures are anatomical features in different species that are similar because they were inherited from a common ancestor. These structures may have different functions in the species where they are found, but their similar underlying structure indicates a shared evolutionary origin.
- Example: The forelimbs of humans, bats, whales, and cats are homologous structures. Despite their different functions—grasping in humans, flying in bats, swimming in whales, and walking in cats—their bone structures are similar. This similarity indicates that these species share a common ancestor that had a similar limb structure.
- Evolutionary Significance: Homologous structures provide strong evidence for evolutionary relationships and are used to construct phylogenetic trees. The presence of homologous structures suggests that species with these traits are closely related.
Analogous Structures:
- Definition: Analogous structures are features in different species that serve similar functions but did not arise from a common ancestor. Instead, they evolved independently in different lineages as adaptations to similar environmental challenges.
- Example: The wings of birds, bats, and insects are analogous structures. While all these wings serve the function of flight, they evolved independently in each lineage. Birds’ wings are modified forelimbs with feathers, bats’ wings are also modified forelimbs but with a membrane stretched between the fingers, and insect wings are extensions of the exoskeleton.
- Evolutionary Significance: Analogous structures result from convergent evolution, where different species evolve similar traits independently, often due to similar selective pressures. Analogous structures do not indicate a close evolutionary relationship.
Summary:
- Homologous structures arise from a common ancestor and may have different functions but similar structures.
- Analogous structures have similar functions but arise independently in different species and do not indicate a close evolutionary relationship.
Understanding the difference between homologous and analogous structures is crucial in evolutionary biology for accurately interpreting the evolutionary relationships and the adaptive strategies of organisms.
How do scientists determine the evolutionary relationships among species?
Scientists determine the evolutionary relationships among species through a combination of comparative anatomy, molecular data, and fossil evidence. The process involves analyzing similarities and differences in physical structures, genetic sequences, and evolutionary history. Here are the key methods used:
- Comparative Anatomy:
- Scientists compare the physical structures (morphology) of different organisms to identify homologous structures, which indicate a common ancestry. For example, the similarity in bone structure in the forelimbs of vertebrates suggests they share a common ancestor.
- Analogous structures, which arise from convergent evolution, are also analyzed to understand how different species adapted to similar environments independently.
- Molecular Data:
- DNA Sequencing: Comparing DNA sequences between species is one of the most precise methods for determining evolutionary relationships. Closely related species will have more similar DNA sequences, reflecting their recent common ancestry.
- Protein Analysis: Scientists also compare the amino acid sequences of proteins across different species. Similarities in protein sequences can indicate evolutionary relationships.
- Molecular Clocks: The concept of a molecular clock is used to estimate the time of divergence between species. By comparing the number of genetic mutations that have accumulated over time, scientists can estimate how long ago two species shared a common ancestor.
- Fossil Evidence:
- Fossils provide a historical record of life on Earth and can show the progression of evolution in a lineage. By studying the morphology of fossils and their stratigraphic position (which layer of rock they are found in), scientists can infer evolutionary relationships and the timing of divergence events.
- Transitional fossils, which show intermediate forms between ancestral and descendant species, are particularly important for understanding evolutionary transitions.
- Phylogenetic Analysis:
- Scientists use phylogenetic trees to represent the evolutionary relationships among species. These trees are constructed using data from comparative anatomy, molecular studies, and fossils. The trees illustrate how species are related and how they have diverged from common ancestors.
- Cladistics: A method used to create phylogenetic trees, cladistics groups species based on shared derived characteristics (traits that evolved in the common ancestor of the group and are present in all its descendants).
- Biogeography:
- The geographic distribution of species is also used to infer evolutionary relationships. Closely related species are often found in proximity to each other, suggesting they evolved from a common ancestor in that region. Biogeographical studies can provide insights into how species have spread and diversified over time.
- Behavioral and Ecological Data:
- Behavioral traits, such as mating rituals or feeding behaviors, can also provide clues about evolutionary relationships. Ecological roles and adaptations to specific environments are studied to understand how species have evolved to occupy their niches.
By integrating data from these various sources, scientists can build a comprehensive picture of the evolutionary relationships among species. Advances in molecular biology, particularly DNA sequencing, have greatly enhanced our ability to accurately determine these relationships.
What are the different types of speciation?
Speciation is the evolutionary process by which populations evolve to become distinct species. There are several types of speciation, each of which describes a different mechanism through which new species arise. The main types of speciation are:
- Allopatric Speciation:
- Definition: Occurs when a population is geographically isolated from other populations of the same species. Over time, the isolated population accumulates genetic differences due to mutation, natural selection, and genetic drift. If the geographic barrier remains for long enough, the two populations may diverge into distinct species.
- Example: The formation of new species on islands, such as the Galápagos finches, is a classic example of allopatric speciation. Each island population evolved independently, leading to the development of unique species.
- Sympatric Speciation:
- Definition: Occurs when a new species arises within the same geographic area as the parent population. This can happen due to ecological differences, genetic mutations, or behavioral changes that lead to reproductive isolation, even though the populations are not physically separated.
- Example: Sympatric speciation is observed in some fish species in African lakes, where different populations specialize in different types of food or habitat, eventually leading to reproductive isolation and the formation of new species.
- Parapatric Speciation:
- Definition: Occurs when populations are partially isolated geographically and have a narrow zone of overlap where they can still interbreed. However, different selective pressures in distinct environments lead to divergence, and over time, reproductive barriers develop, resulting in speciation.
- Example: Grass species growing on contaminated soil near mines may undergo parapatric speciation. Plants in the contaminated soil develop tolerance to heavy metals, while those in uncontaminated soil do not, leading to the formation of two distinct species.
- Peripatric Speciation:
- Definition: A special case of allopatric speciation, where a small population becomes isolated at the edge of a larger population. Genetic drift and natural selection in the smaller population can lead to rapid divergence and the formation of a new species.
- Example: The speciation of polar bears from brown bears is an example of peripatric speciation. A small population of brown bears became isolated in the Arctic, leading to the evolution of polar bears.
- Hybrid Speciation:
- Definition: Occurs when two different species interbreed and produce hybrid offspring that are reproductively isolated from both parent species. This process can lead to the formation of a new species if the hybrids can establish a stable population.
- Example: Some species of sunflowers are believed to have arisen through hybrid speciation, where hybrids between two different sunflower species became reproductively isolated and evolved into a new species.
- Artificial Speciation:
- Definition: A form of speciation that occurs due to human intervention, where humans selectively breed individuals with certain traits, eventually leading to the formation of new species.
- Example: The domestication of animals and plants, such as dogs and cultivated crops, is an example of artificial speciation. Selective breeding has led to the development of new species with traits that are advantageous to humans.
Each type of speciation involves different mechanisms and environmental conditions, but all result in the formation of new species, contributing to the diversity of life on Earth.
What is adaptive radiation, and can you provide an example?
Adaptive radiation is an evolutionary process in which a single ancestral species rapidly diversifies into a large number of new species, each adapted to a different ecological niche. This process typically occurs when a species colonizes a new environment with diverse habitats and resources or when a major extinction event opens up ecological opportunities for the surviving species.
Key Features of Adaptive Radiation:
- Rapid Speciation: Adaptive radiation involves the rapid formation of many new species in a relatively short period of time.
- Ecological Niches: The new species that arise from adaptive radiation each occupy different ecological niches, meaning they exploit different resources or have different roles in the ecosystem.
- Morphological and Behavioral Diversity: The species that result from adaptive radiation often exhibit a wide range of morphological and behavioral adaptations to their specific environments.
Example:
The Darwin’s finches of the Galápagos Islands are one of the most famous examples of adaptive radiation. When Charles Darwin visited the Galápagos in the 19th century, he observed a group of finches that had diversified into a variety of species, each with a unique beak shape and size. These beak variations allowed different finch species to exploit different food sources, such as seeds, insects, and fruit. For instance:
- The ground finches have thick, strong beaks suited for cracking seeds.
- The cactus finches have long, pointed beaks adapted to feeding on cactus flowers and fruit.
- The woodpecker finch uses its beak to pry insects from tree bark, sometimes using tools like sticks to extract its prey.
This diversification of finches from a common ancestor allowed them to thrive in the different environments of the Galápagos Islands, demonstrating the process of adaptive radiation.
Adaptive radiation is a key mechanism in the diversification of life and has occurred in many different groups of organisms throughout Earth’s history, including mammals after the extinction of the dinosaurs and cichlid fish in the lakes of East Africa.
How does genetic drift differ from natural selection?
Genetic drift and natural selection are both mechanisms of evolution, but they operate in different ways and have different effects on the genetic composition of populations.
Genetic Drift:
- Definition: Genetic drift refers to random changes in the frequency of alleles (variations of a gene) within a population. These changes are due to chance events rather than selective pressures. Genetic drift is most significant in small populations, where random events can have a larger impact on allele frequencies.
- Mechanism: Genetic drift occurs purely by chance. For example, if a small population experiences a natural disaster that randomly eliminates individuals regardless of their genetic traits, the surviving population may have a different genetic makeup than the original population.
- Outcome: Over time, genetic drift can lead to the loss of genetic diversity within a population and can cause certain alleles to become fixed (reach a frequency of 100%) or lost entirely. Unlike natural selection, genetic drift does not necessarily lead to adaptations that increase an organism’s fitness.
- Examples: The bottleneck effect and the founder effect are examples of genetic drift. In the bottleneck effect, a large portion of a population is suddenly reduced in size, and the surviving population has a different allele frequency than the original population. In the founder effect, a small group of individuals colonizes a new area, and the genetic composition of this group may differ from the original population.
Natural Selection:
- Definition: Natural selection is the process by which certain traits become more common in a population because they provide a survival or reproductive advantage. Individuals with advantageous traits are more likely to survive and reproduce, passing those traits on to the next generation.
- Mechanism: Natural selection is driven by environmental pressures, such as predation, competition, and climate. Traits that enhance an organism’s ability to survive and reproduce in a particular environment are more likely to be passed on, while less advantageous traits become less common.
- Outcome: Natural selection leads to adaptations—traits that improve an organism’s fitness in its environment. Over time, populations become better suited to their environments through the process of natural selection.
- Examples: The evolution of antibiotic resistance in bacteria is an example of natural selection. Bacteria with mutations that confer resistance to antibiotics are more likely to survive and reproduce, leading to a population dominated by resistant strains.
Summary:
- Genetic drift is random and can lead to changes in allele frequencies due to chance, especially in small populations. It does not necessarily lead to adaptations.
- Natural selection is a non-random process that favors traits that enhance survival and reproduction, leading to adaptations in the population.
Both genetic drift and natural selection contribute to the evolution of species, but they operate through different mechanisms and have different effects on genetic variation within populations.
What role do mutations play in evolution?
Mutations are changes in the DNA sequence of an organism’s genome. They are the ultimate source of genetic variation, which is essential for evolution. Without mutations, there would be no new genetic material for natural selection to act upon, and evolution would not occur.
Types of Mutations:
- Point Mutations: Changes in a single nucleotide base in the DNA sequence. These can be silent (no change in the protein), missense (a different amino acid is produced), or nonsense (a stop codon is created, truncating the protein).
- Insertions and Deletions: The addition or loss of nucleotides in the DNA sequence. These can lead to frameshift mutations, which alter the reading frame of the gene and often result in nonfunctional proteins.
- Gene Duplications: Entire genes or sections of the genome are duplicated, providing raw material for the evolution of new genes with potentially new functions.
- Chromosomal Mutations: Large-scale changes in the structure or number of chromosomes, such as inversions, translocations, or aneuploidy (extra or missing chromosomes).
Role of Mutations in Evolution:
- Source of Genetic Variation:
- Mutations introduce new alleles into a population, increasing genetic diversity. This variation is the raw material for evolution, as it provides the potential for new traits to emerge.
- Most mutations are neutral, having no effect on an organism’s fitness. However, some mutations can be beneficial, conferring an advantage in a particular environment, or deleterious, reducing an organism’s fitness.
- Driving Adaptation:
- Beneficial mutations that enhance an organism’s ability to survive and reproduce may increase in frequency in the population through natural selection. Over time, these advantageous traits become more common, leading to adaptation to the environment.
- For example, a mutation that allows bacteria to resist an antibiotic can quickly spread through a population, leading to the evolution of antibiotic-resistant strains.
- Speciation:
- Mutations can contribute to the divergence of populations, leading to the formation of new species. For example, mutations that affect reproductive compatibility, such as changes in mating behavior or timing, can lead to reproductive isolation and eventually speciation.
- Evolutionary Innovation:
- Gene duplications and other large-scale mutations can lead to the evolution of entirely new genes or gene functions. These innovations can result in the development of new structures, metabolic pathways, or behaviors, contributing to the diversity of life.
- For instance, the evolution of vertebrate hemoglobin, a protein that carries oxygen in the blood, involved gene duplication and subsequent mutations that allowed the protein to take on a new function.
- Molecular Evolution:
- Mutations in regulatory regions of DNA can alter gene expression patterns, leading to changes in the timing, location, or amount of gene product produced. These regulatory mutations can have significant evolutionary consequences, leading to the development of new traits and forms.
Conclusion:
Mutations are essential to the process of evolution, as they provide the genetic variation that fuels evolutionary change. While most mutations may be neutral or harmful, the occasional beneficial mutation can lead to significant evolutionary advancements, shaping the diversity of life on Earth.
What is the difference between microevolution and macroevolution?
Microevolution and macroevolution are two scales of evolutionary processes, describing changes that occur within populations and the broader patterns that result from these changes over long periods of time.
Microevolution:
- Definition: Microevolution refers to small-scale changes in allele frequencies within a population over a short period of time. These changes are driven by mechanisms such as natural selection, genetic drift, gene flow, and mutations.
- Focus: Microevolutionary studies focus on the processes that cause populations to evolve, such as how certain traits become more common or rare in a population.
- Examples:
- The development of antibiotic resistance in bacteria is a macroevolutionary process. As certain bacteria acquire mutations that confer resistance, these bacteria survive and reproduce, leading to an increase in the frequency of the resistance allele in the population.
- Changes in the coloration of peppered moths during the Industrial Revolution in England are another example. The dark-colored moths became more common due to their camouflage in soot-covered environments, demonstrating microevolution through natural selection.
Macroevolution:
- Definition: Macroevolution refers to large-scale evolutionary changes that occur over long periods of time, leading to the emergence of new species, genera, families, and higher taxonomic groups. Macroevolutionary patterns are the result of the accumulation of microevolutionary changes.
- Focus: Macroevolutionary studies focus on broad patterns in the history of life, such as the origin of new species (speciation), mass extinctions, adaptive radiations, and the evolution of major body plans.
- Examples:
- The evolution of mammals from reptile-like ancestors over millions of years is an example of macroevolution. This process involved the gradual accumulation of changes in anatomy, physiology, and behavior, leading to the emergence of a new class of animals.
- The diversification of flowering plants during the Cretaceous period, leading to the wide variety of plant forms we see today, is another example of macroevolution.
Relationship Between Microevolution and Macroevolution:
- Microevolutionary processes, such as natural selection and genetic drift, provide the raw material for macroevolution. Over long periods of time, the accumulation of small genetic changes can lead to significant evolutionary shifts, resulting in the formation of new species and higher taxonomic groups.
- While microevolution focuses on the genetic and ecological dynamics within populations, macroevolution examines the long-term consequences of these changes, including the origins of new species and the patterns of diversity and extinction seen in the fossil record.
Conclusion:
Microevolution and macroevolution are interconnected aspects of the same evolutionary process. Microevolution describes the changes within populations that lead to genetic diversity, while macroevolution looks at the larger-scale patterns that result from the accumulation of these changes over geological time. Together, they provide a comprehensive understanding of how life evolves on Earth.
How does sexual selection influence evolution?
Sexual selection is a type of natural selection that arises from differences in mating success among individuals. It plays a significant role in shaping the evolution of species by influencing traits that affect an organism’s ability to attract mates and successfully reproduce.
Types of Sexual Selection:
- Intersexual Selection (Mate Choice):
- Definition: Occurs when individuals of one sex (usually females) choose mates based on specific traits. These traits may be indicators of genetic fitness, health, or the ability to provide resources.
- Examples:
- Peacock’s Tail: The elaborate and colorful tail feathers of male peacocks are a result of intersexual selection. Females prefer males with larger and more vibrant tails, leading to the evolution of this exaggerated trait.
- Bird Songs: In many bird species, males with more complex or louder songs are more successful in attracting mates, as these traits may signal good health or superior genes.
- Intrasexual Selection (Competition):
- Definition: Occurs when individuals of the same sex (usually males) compete with each other for access to mates. Traits that enhance success in these contests, such as physical strength or weaponry, are favored.
- Examples:
- Antlers in Deer: Male deer use their antlers in battles with other males to win access to females. Larger and stronger antlers increase a male’s chances of winning these contests and reproducing.
- Body Size in Elephant Seals: Male elephant seals engage in intense physical fights to establish dominance and control over a group of females. Larger males with greater strength are more likely to win these battles and secure mates.
Consequences of Sexual Selection:
- Sexual Dimorphism: Sexual selection often leads to the evolution of sexual dimorphism, where males and females of a species exhibit different physical characteristics. For example, in many bird species, males are more colorful than females, a result of intersexual selection.
- Runaway Selection: In some cases, sexual selection can lead to the evolution of traits that are extremely exaggerated, to the point where they may even be detrimental to survival. For example, the peacock’s tail is so large and elaborate that it can hinder the male’s ability to escape predators, yet it persists due to female preference.
- Evolution of Behavior: Sexual selection also influences the evolution of behaviors, such as courtship displays, mating dances, and vocalizations, that increase an individual’s attractiveness to potential mates.
Impact on Evolution:
- Genetic Diversity: Sexual selection can maintain or even increase genetic diversity within a population by favoring multiple traits or strategies. For example, in some species, both dominant and sneaky male strategies coexist, leading to a balance of traits in the population.
- Speciation: Sexual selection can contribute to speciation by driving the divergence of mating preferences or reproductive behaviors. If different populations develop distinct mating preferences, they may become reproductively isolated and evolve into separate species.
- Adaptation: While sexual selection often favors traits that increase reproductive success, these traits can sometimes conflict with natural selection. For instance, a trait favored by sexual selection may reduce an individual’s survival chances, leading to a balance between sexual and natural selection pressures.
Conclusion:
Sexual selection is a powerful force in evolution, shaping the development of physical traits, behaviors, and reproductive strategies. It contributes to the diversity of life by driving the evolution of characteristics that enhance mating success, sometimes leading to remarkable adaptations and the emergence of new species.
These explanations cover fundamental topics in evolutionary biology and provide a solid foundation for understanding the processes that drive the diversity of life on Earth.
Read More Articles
- Indirect Methods for Measurement of Large Distances
- Measurement of Length: Principles, Techniques, & Applications
- Range of Variation of Length in Measurement with Perfect Examples
- Understanding the Order of Magnitude with Perfect Examples
- The Advantages of SI Units with Proper Explanation
- Fundamental and Supplementary Units with Proper Explanation
- System of Units in Physics with Proper Explanation
- Unit of Time in Physics with Proper Explanation
- Unit of Length in Physics with Proper Explanation
- Unit of Mass in Physics: An In-Depth Exploration
- Characteristics of Standard Units in Physics with Proper Explanation
- Fundamental Units and Derived Units in Physics with Proper Explanation
- Units for Measurement in Physics with Proper Explanation
- The Crucial Role of Measurement in Physics with Proper Explanation
- The Nature of Physical Laws with Proper Explanation
- Fundamental Forces in Nature with Detailed Explanation
- Physics in Relation to Technology with Proper Explanation
- Physics in Relation to Society with Proper Explanation
- Physics in Relation to Science with Proper Explanation
- Physics: The Scope and Excitement of Physics
- Physics And Its Fundamentals With Good Explanations
- The Application of Quantum Mechanics
- An Overview of Quantum Mechanics
- A Comprehensive Guide to String Theory: Unifying Physics